The Artificial Leaf: Copying Nature to Fight Climate Change
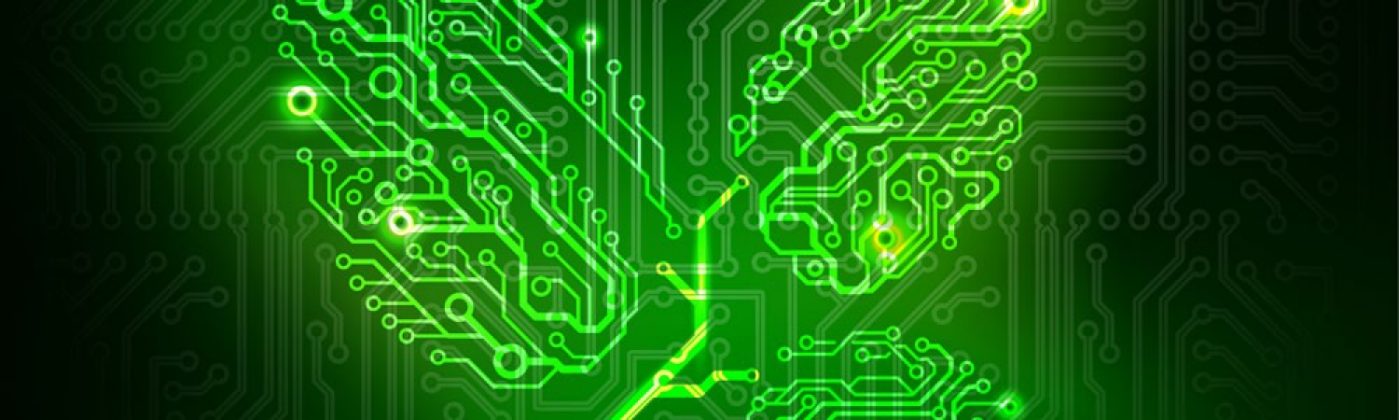
Every plant, animal, and person owes their life to one sequence of chemical reactions: photosynthesis. The process, which converts water and carbon dioxide into food using sunlight, first evolved in cyanobacteria more than 2 billion years ago.
That’s right. Plants weren’t the first organisms to develop photosynthesis, though they are better known for it. Cyanobacteria originally filled the atmosphere with photosynthesis’s gaseous by-product, oxygen (O2), which set the stage for virtually all plant and animal life.
As beneficiaries of photosynthesis, humans depend on plants in a sort of carbon seesaw. Plants take in CO2 and release O2. They store that carbon as sugar. Hanging vines, grass, and trees all grow by pulling carbon atoms out of the air. We do the reverse, taking in O2 and releasing CO2. Finally, everything we eat completes the handoff: human plant (or the animal who already did), human exhales, plant stores carbon, and the cycle continues.
This seesaw is part of the much broader carbon cycle that has affected the radiation balance of our planet. Cutting down huge swaths of forests and the burning of carbon-based fossil fuels cause the levels of CO2, a major greenhouse gas, to rise. And plants on Earth along with other natural parts of the carbon cycle can’t restore the balance on their own.
But what if we could copy what plants do—grab some of that excess CO2 to make fuels sustainably, instead of relying so heavily on fossilized carbon?
“Artificial photosynthesis is a really attractive approach,” says Jillian Dempsey, a professor of chemistry at the University of North Carolina, Chapel Hill. “You’re able to store the energy of the sun in the bonds of [molecules].”
At a large enough scale, such sun-powered processes could give us enough energy-storing molecules to make fuels out of thin air and water like plants and cyanobacteria do.
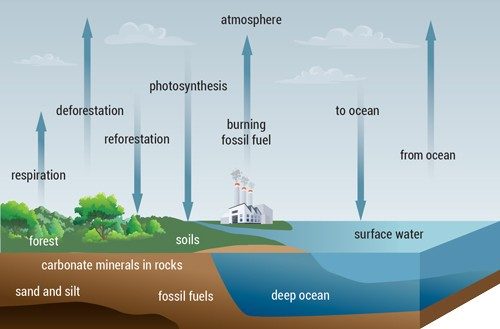
Plants make it look easy
Although it’s tempting to write photosynthesis off as a simple reaction—CO2 and H2O come in, a leaf makes its food, and O2 heads out—the chemistry occurring is surprisingly complex. It’s a dance of protons, electrons, and biological machinery that had millions of years to evolve. That machinery severs the strong bonds in H2O and CO2 to build more complex molecules, such as glucose (C6H12O6).
What the reaction equations don’t show is what spurs the reactions: the catalysts, which lower the activation energy of chemical transformations. Since they lower the activation energy needed to break and form just the right bonds, the catalysts of photosynthesis are basically calling the shots. Making complex sugars out of CO2 wouldn’t be feasible without them. And while nature has had billions of years to perfect the process, researchers have succeeded in building small devices that not only mimic natural photosynthesis, but also beat it in just a few decades.
Photosynthesis 2.0
Let’s take a look at the role catalysis plays in photosynthesis. First, plants absorb sunlight in their chloroplasts, which are organelles found in plant and algae cells. Inside the chloroplasts are chlorophyll pigments that absorb mostly red and blue, but not green, wavelengths of light (thus their color). This absorbed energy powers the plant’s chemical reactions.
Artificial photosynthesis needs its own version of chlorophyll. To find substances to act like chlorophyll in an artificial leaf, scientists are testing natural pigments, synthetic dyes, or other materials, such as semiconductor films and particles that absorb visible light.
In plants, once light is absorbed, a complicated chain of biological catalysts (i.e., enzymes) carries out reactions in a precise order to create fuels such as glucose. One sequence of reactions, called photophosphorylation, creates biological energy—using the energy of sunlight and phosphorus to turn a plant’s adenosine diphosphate (ADP) into the more energetic adenosine triphosphate (ATP) molecules. The other, called the Calvin cycle, uses that ATP to build complex molecules like glucose from simple CO2.
Every step in both operations relies on unique enzymes. But chemists can’t simply steal those exact enzymes, glue them onto some solar panels, and expect them to make fuels. Enzymes can only maintain their structure and reactivity under the specific biological conditions found inside cells. Plus, scientists want to do even better than those natural enzymes.
“The goal of artificial photosynthesis is to be inspired by some of those natural phenomena, but design it with components the plant doesn't have available to it, like inorganic catalysts and semiconductors, and make a similar process that's much more efficient,” says Josh Spurgeon, a solar fuels researcher at the Conn Center for Renewable Energy Research at the University of Louisville.
Rather than making glucose, researchers want to produce fuels, such as ethanol, that our energy-driven technologies can use. Alternatively, some artificial-leaf technologies don’t require CO2 but rather take in water to produce hydrogen fuel (H2).
The simplest fuel
A fuel cell’s power comes from the combustion of H2, a process that produces no carbon emissions—its only emission is H2O. Today, most H2 is produced from methane (CH4) in a process that emits CO2. If scientists could figure out how to make H2 using sunlight in an affordable way, hydrogen fuels would be more sustainable.
To do this with an artificial leaf, it would have to carry out the oxidation and reduction reactions required to make H2 from H2O, or “water splitting.” Like photosynthesis, the reactions seem straightforward on paper.
2H2O → O2 + 4H+ + 4e-
4H+ + 4e- → 2H2
“If I just put a cup of water out in the sunshine, it’s not going to spontaneously turn into hydrogen and oxygen,” says Dempsey, who designs catalysts for solar-fuel production. With an enthalpy of formation of -285.83 kJ/mol, H2O doesn’t easily produce H2 (∆Hf = 0 kJ/mol).
Catalysts like iron oxide and platinum are particularly effective at O2 and H2 reactions. For the oxygen half of the reaction, Spurgeon says, designing an inexpensive, efficient, and stable catalyst has been tough. “If you do this in acid, where a lot of water electrolysis is most efficient, there's really only a couple of materials that work,” he says. Iridium oxide is commonly used since it’s the most effective. But iridium is also one of the rarest elements on Earth.
Spurgeon’s lab is working to replace iridium with Earth-abundant alternatives, such as tungsten or tantalum.
Building with naturally abundant elements makes technologies less expensive overall. The element rhodium, for example, is often used in car catalytic converters and some water-splitting designs, but it’s rare. “So choosing something like cobalt over rhodium has a clear economic advantage in terms of making these cost-effective solutions,” says Dempsey.
By studying new elements and crystalline hybrids of multiple catalysts, scientists are pushing the boundaries of what’s possible for artificial photosynthesis. “Catalysis gives us access to seemingly impossible chemical transformations,” Dempsey says.
Making carbon fuels
Ethanol, (C2H5OH), typically makes up 10% of the gas you pump into most cars. Some flex-fuel cars can handle up to 85% C2H5OH.
Today, most ethanol in the US comes from fermenting crops, which rely on a lot of land, water, and energy from fossil fuels to grow. This is where artificial leaf researchers come in. They are engineering catalysts to produce ethanol and butanol (C4H9OH) in a more sustainable way. Butanol’s uses are similar to ethanol’s.
“To build carbohydrates or ethanol or butanol,” says Dempsey, “we need to carefully choreograph how the protons and the electrons are being reassembled.” Turning a couple of CO2 molecules into C2H5OH requires shuffling around 12 H+ [protons] and 12 electrons.
2CO2 + 12H+ + 12e- → C2H5OH + 3H2O
What’s extraordinary is that these chemical choreographies work. Light, CO2, and water go in; fuel comes out. And in some ways, chemists have designed catalysts to make these fuels more efficiently than plants produce sugars.
“It turns out that natural photosynthesis isn’t actually that efficient of a process,” Dempsey says. Only about 1% of the solar energy that hits a plant turns into fuel energy. The efficiency of artificial leaf technologies can exceed 20%.
This doesn’t mean artificial photosynthesis copies natural photosynthesis in all aspects though. Plants are less efficient, but they make more complex fuels with greater precision than artificial devices. “Some of these systems that are showing promise make a whole slew of stuff—12 different products mixed together,” Dempsey says. Molecules like methane, carbon monoxide, formic acid, and ethylene could form in undesirable side reactions.
Ideally, all electrons would funnel onto the correct atoms or molecules. But CO2 and its reduction by-products end up competing with each other and hydrogen. “There are a lot of ways that chemistry can go,” says Spurgeon. “You want to keep the hydrogen evolution low and get enough of those carbons next to each other that you can form carbon-carbon bonds and make multicarbon products.” In other words, it’s tough to stop the reaction at C-C formation—it wants to go to CO2 or other energetically favorable products.
Currently, the major challenge in catalyst research involves designing materials that speed up specific reactions and make only the products we want. “We have gotten to the point where scientists have done enough preliminary studies to know that what we are proposing is achievable,” Dempsey says.
Butanol
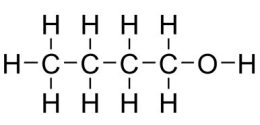
Ethanol
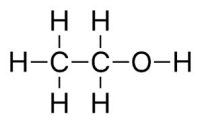
Making an artificial leaf
So, what would an artificial leaf device actually look like?
“It basically looks like this sandwich structure—the catalyst layers are sandwiching the photoabsorber,” says Peter Agbo, a staff scientist at Lawrence Berkeley National Laboratory who works with the Joint Center for Artificial Photosynthesis.
Existing devices are also small. A hydrogen device with 12.6% efficiency that Agbo recently built was less than one inch across. For artificial photosynthesis to become practical, it needs to produce fuels at a large scale to compete with the world’s existing energy supply of relatively inexpensive and abundant fossil fuels.
The end goal for photoabsorbers is a material that is efficient, stable, and durable. Spurgeon feels that right balance will come from a hybrid of technologies--using stable microparticles like silicon, for instance, in conjunction with efficient III-V semiconductors, such as indium gallium nitride.
A company called Twelve recently developed a “shiny black leaf that eats carbon.” Their products turn CO2 into formic acid (H2CO2). Other labs focus on making ethanol. But the efficiency of all of these products lags behind hydrogen devices. (A state-of-the-art combination of photovoltaic devices and hydrogen evolution--not a stand-alone [hyphen added] artificial-leaf [hyphen added] device--has reached well over 30% total efficiency using expensive materials.)
So, scaling up artificial photosynthesis is still far off, but it’s moving along.
“We’ve identified the fundamental scientific challenges,” Dempsey says. “We’re learning how to put the different pieces together.”
What might ultimately get the technology across the finish line is another turn back to nature—but this time, instead of just copying it, scientists want to use it. Engineers from the University of California, Berkeley, for example, recently combined nanoparticles with living nonphotosynthetic bacteria.
Their microbe of choice, Moorella thermoacetica, naturally reduces CO2 to make a small amount of acetic acid (CH3COOH). When the researchers fed the bacteria tiny clusters of gold atoms and exposed them to sunlight, the gold clusters were able to pull electrons from the amino acid cysteine and send them to the microbe’s enzymes. The electrons interacted with the bug’s enzymes, which spurred the bacteria to make much more acetic acid from CO2 than they normally would have. The acetic acid can then be used to make fuels and other valuable chemicals.
It will take a lot of time and money before artificial photosynthesis can compete with fossil fuels. But the needed investment won’t come close to the societal cost of climate change. A recent survey of more than 2,000 economists projected the economic damages from climate change will reach $1.7 trillion per year by 2025 and roughly $30 trillion per year by 2075.
Artificial photosynthesis could inch us back toward a better balance on the planet’s carbon seesaw.
References
Hisatomi, T.; Kubota, J.; Domen, K. Recent Advances in Semiconductors for Photocatalytic and Photoelectrochemical Water Splitting. Chem. Soc. Rev. 2014, 43(22), 7520-7535. https://pubs.rsc.org/en/content/articlelanding/2014/cs/c3cs60378d [accessed Aug 2021].
Kistler, T. A.; Danilovic, N.; Agbo, P. A Monolithic Photoelectrochemical Device Evolving Hydrogen
in Pure Water. J. Electrochem. Soc. 2019, 166(13), H656. https://iopscience. iop.org/article/10.1149/2.1151913jes [accessed Aug 2021].
Yang, P. Liquid Sunlight: The Evolution of Photosynthetic Biohybrids. Nano Lett. 2021, 21(13), 5453−5456. https://pubs.acs.org/doi/pdf/10.1021/acs. nanolett.1c02172 [accessed Aug 2021].
This story was originally published in the October 2021 issue of ChemMatters Magazine.